Life Support Devices in Intensive Care
From: University of Michigan | By: Robert H. Bartlett, M.D.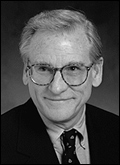
On June 5, 2000, TE (patient's name withheld for anonymity) had just completed his third year at Indiana University. He was in excellent health, a gymnast and captain of the IU cheerleading squad. On June 7, 2000, he was in septic shock, comatose, in renal failure, and on the mechanical ventilator for severe acute respiratory distress syndrome, dying of meningococcal sepsis. With five organ systems failing, TE was referred to the Extracorporeal Life Support Service at the University of Michigan. He was too unstable for conventional transport, so the ECLS team went to the referral hospital 200 miles away, cannulated his heart through vessels in the neck, established a venoarterial cardiopulmonary bypass, and transported him back to the Surgical Intensive Care Unit at University Hospital in Ann Arbor. For the next 20 days, TE's life was maintained by mechanical devices. In the ICU he was sustained by more artificial organs (heart, lungs, kidneys and intestine) than native organs, all designed to keep his brain alive and healthy. We will return to TE's story later.
The use of man-made devices to replace the function of vital organs is only about 50 years old. The transition from basic science research to routine clinical application has proceeded so quickly that it is now commonplace, even to the lay public. It is considered routine to have an artificial heart valve, pacemaker, hip joint, or artery, or to be on an artificial kidney or mechanical ventilator, or on completely intravenous feedings. Organ replacement makes it possible to provide a bridge to organ recovery or organ transplantation. Millions of people have had their lives extended and improved by artificial organs.
Consider the challenge that faced those researchers who developed devices that replace organ function. To substitute for vital organ functions requires not only the basic science of bioengineering, but also a thorough understanding of the basic science related to the native organ itself. What has been done seems quite simple in retrospect, but one need only consider the challenge of developing the next generation of artificial organs to see the complexity of the project. In this feature I will focus on the short-term use of artificial organs in intensive care, from heart and lung to liver function, because it provides the best examples of the application of basic science to this clinical discipline.
Bioengineering and the cardiopulmonary bypass
Consider first the bioengineering research necessary to replace heart and lung function. The scientist who first solved this problem was a Philadelphia surgeon named Dr. John Gibbon. Dr. Gibbon's clinical incentive was a young woman who died of a massive pulmonary embolism while under his care. He recognized that the fatal problem was a temporary mechanical obstruction of pulmonary blood flow, and a mechanical device that substituted for heart and lung function for a short time could have solved the problem.
Dr. Gibbon and his wife, Mali, developed such a device. Their heart-lung machine used a roller pump to substitute for the heart, and it exposed blood directly to oxygen gas filmed over screens as a substitute for the lung. They tested the device on the bench, then in animals. The device drained blood from the right atrium and pumped it into the aorta, a process the Gibbons called cardiopulmonary bypass. Exposure of blood to the rubber, metal, and glass components of the system resulted in clotting, however. Although heparin could inhibit some of the clotting, platelet aggregates still formed on the surface and embolized into the patient. In addition, the physiology associated with cardiopulmonary bypass resulted in hypotension (low blood pressure), metabolic acidosis (low blood pH) and thrombocytopenia (low platelets). Although the heart-lung machine was not without its difficulties or its dangers, patients could tolerate it for a few hours. Cardiopulmonary bypass led to the entire field of cardiac surgery.
One of the pioneers of cardiac surgery was Robert E. Gross, chief of surgery at the Boston Children's Hospital. The mortality for repair of complicated cardiac defects in children was 50 percent, although it was clear that if the children could survive just a few days, they would ultimately recover. As a naïve junior resident I asked Dr. Gross if we could use the heart-lung machine for a few days to sustain the life of these patients until their native heart recovered. He observed that the heart-lung machine was itself a lethal instrument if used for more than an hour or two, but he encouraged our research to extend the use of the heart-lung machine for longer periods.
This brings us to the first consideration of artificial organs in intensive care: prolonged substitution for the heart and lung and endothelium (the lining of the blood vessels). A modified heart-lung machine would have to include a pump, some way of controlling thrombogenicity (the production of blood clots) and a blood gas exchange device commonly called an oxygenator. It soon became apparent that the lethal component of the original heart-lung machine was the oxygenator. Direct exposure of the blood to gas certainly results in gas exchange but also activates and denatures plasma protein, platelets and white blood cells; disrupts the integrity of red blood cell membranes; and incorporates micro-bubbles in the flowing blood.
How to exchange gas in blood without these complications? In the 1960s, we and others developed membrane oxygenators in which a gas-permeable membrane of silicone rubber was substituted between the blood and the gas interface. There were some problems to resolve. The efficiency of the device to oxygenate venous blood, particularly that in the center of the flow path, had to be improved. The membrane lung malfunctions very much like the human lung, so problems could be recognized and solved. The heart-lung machine for cardiac surgery has a large blood reservoir, which requires a large amount of heparin for anticoagulation. To eliminate the reservoir it was necessary to modify the roller pump to permit its continuous use but to avoid direct suction on the venous drainage catheter.
Phil Drinker, an engineer at the Brigham Hospital on loan from MIT, and I undertook to design an oxygenator, then to use it for prolonged extracorporeal circulation in the laboratory. Phil is shown here with a dog that has been on partial bypass for five days. Common enough nowadays, but pretty exciting stuff in 1966. We learned that the amount of heparin required to prevent thrombosis in this very simplified membrane oxygenator circuit was much less than that commonly used for cardiac surgery, which resulted in our ability to maintain perfusion for days without any bleeding from the animal. With this preparation we were able to study the physiologic response to long-term perfusion and found it quite different than the metabolic response to cardiopulmonary bypass with conventional equipment.
With the roller pump, the blood flow is constant and does not pulse. We needed to understand the physiologic response to chronic non-pulsatile flow. Many scientists investigated this problem, including Eugene Bernstein, whose studies are considered definitive. It is now clear that as long as the total flow is normal, there is no difference in response to perfusion with pulsatile or non-pulsatile flow, except at abnormally low levels of flow, when there is a slight protective effect of maintaining pulsatile flow. The problem is simply addressed by maintaining adequate levels of blood flow, resulting in stable hemodynamic and metabolic physiology during long periods of extracorporeal circulation. Other laboratories were working on this problem at the same time. The most successful was the laboratory of Ted Kolobow at the NIH.
Bioengineering in clinical practice
The first successful clinical use of this technology was carried out by San Francisco surgeon Don Hill, who brought his modified heart-lung machine to treat a patient in Santa Barbara. This patient, treated in 1971, eventually recovered. As in the operating room, venous blood is drained from the right side of the heart, pumped through an artificial lung, in this case a membrane lung, and back into the arterial circulation of the patient. In lambs we used the carotid artery and jugular vein, and soon we learned that the collateral circulation was adequate to do the same in children.
In 1972 my partner, Al Gazzaniga, and I were faced with a child in cardiogenic shock. This two-year-old boy with profound acidosis (highly acidic pH level in the blood) and low cardiac output was maintained on extracorporeal support for 36 hours, during which time his acidosis cleared, his heart function improved and he eventually recovered. In 1975 we were asked to see a newborn infant with meconium aspiration and persistent fetal circulation in the neonatal ICU. We attached this little girl to our laboratory heart-lung machine. The nurses named this child Esperanza, "hope" in Spanish. After three days on extracorporeal support Esperanza recovered, leading to continued application of this technology to other newborn infants with respiratory and cardiac failure from a variety of problems.
Over the next decade the number of cases grew, and other centers developed the technology. Our ECLS project moved from the University of California-Irvine to the University of Michigan in 1980 and progressed through a series of laboratory research and clinical research studies and seminars to teach other people the technology. This became the standard technique for management of neonatal respiratory failure in 1986. Because the membrane oxygenator featured prominently in the artificial device, the technique became known as Extracorporeal Membrane Oxygenation or ECMO, although it can be described by many other terms, from cardiopulmonary bypass in the operating room to the "Ivox" intravascular membrane oxygenator. We prefer the term Extracorporeal Life Support (ECLS).
In the last ten years prolonged extracorporeal circulation has been extended to children and adult patients with respiratory failure much like TE, described earlier. ECLS for respiratory failure is usually used with venovenous access, draining blood from the inferior venacava (large vein in the abdomen), perfusing it through the membrane lung and reinfusing into the right atrium. The parameters that are monitored are shown in the boxes.
In 2001, we reviewed our experience with our first 1,000 cases with extracorporeal life support. Most of our patients were newborn infants, but there is a steadily increasing application of this technology to children, adults and patients with cardiac failure. The University of Michigan experience in each of these categories, along with the worldwide experience, is maintained in the registry of the Extracorporeal Life Support Organization. More than 20,000 patients have been managed with ECLS with survival rates ranging from 30 percent in cardiac failure and cardiac arrest to 80 percent in neonatal respiratory failure. Considering that the patients who are placed on ECLS are patients like TE who have little chance of survival otherwise, these results continue to be encouraging.
In the ICU, in addition to support for respiratory failure, venoarterial bypass can be used for support of a failing heart, either by itself or in conjunction with intra-aortic balloon pumps and direct left-ventricular or right-ventricular assist devices. Patients in cardiac arrest or profound cardiogenic shock can be bridged to transplantation by a left-ventricular assist device, but implantation is complex and exceptionally expensive. We have taken the approach to put patients in cardiac arrest or profound cardiogenic shock onto ECLS support. In venoarterial mode we support the patient until we are sure that the brain, kidneys and other organs are working well, then implant a left-ventricular assist device and list the patient for cardiac transplantation. Our results with this approach show many patients proceeding from cardiac arrest to cardiac recovery or successful cardiac transplantation. Massive pulmonary embolism patients are particularly rewarding because the survival rate is very good, and this brings Dr. Gibbon's dream full cycle to completion.
Continuous blood filtering
In TE's case we used venoarterial access through the vessels in the neck to provide total cardiac support during his profound septic shock and total respiratory support during his acute respiratory failure, but TE also had acute renal failure. Acute renal failure is a common problem in critical illness, and substitution for the native kidney involves hemodialysis or hemofiltration (the removal of certain elements in blood by filtering). The inventor of hemodialysis for the treatment of renal failure is Dr. Willem Kolff, whose original device was made out of sausage casing and dialyzed the patient's blood against a bath resembling extracellular fluid, which allowed the removal of toxins and fluid. Dr. Kolff's invention proceeded to the development of chronic intermittent hemodialysis for chronic renal failure, ultimately leading to the entire field of renal substitution and transplantation.
In the intensive care unit our interest is specifically in acute renal failure. Twenty years ago, the mortality for acute renal failure in the ICU was 80 percent. In hypermetabolic critically ill patients, intermittent hemodialysis often causes hemodynamic instability, so the common practice was to limit fluid intake, thereby limiting nutrition to these patients. Protein intake was kept low because it was thought that protein feeding would induce urea production, resulting in the need for dialysis. We proposed that starvation itself was a major cause of the mortality in acute renal failure. We presented our studies on metabolic balance and acute renal failure at the American Society of Artificial Internal Organs (ASAIO) meeting in 1983, and there met Dr. Peter Kramer from Germany, who had invented the technique of continuous arterovenous hemofiltration. We first instituted continuous hemofiltration in 1983 as a means of studying nutrition in patients with acute renal failure, and we have been using that technique and studying it ever since.
Continuous hemofiltration involves passing blood through a 30,000 Kd pore size hemofilter, allowing a very large amount of extracellular fluid to come through the hemofilter and replacing it with a slightly smaller amount of fluid concocted to resemble extracellular fluid. This continuous exchange of extracellular fluid clears toxins, removes an unlimited amount of salt and water and provides the ability to feed these patients without limit. Because of the materials in the filter, and because of the continuous controlled exchange, there is no hemodynamic instability. We used hemofiltration to study nutrition in renal failure and demonstrated that patients who were fed their full caloric and protein requirements had much better outcomes than patients who were treated by the conventional technique of protein and caloric restriction. From this we learned that inadequate nutrition accounted for about 30 percent of the mortality in acute renal failure.
Currently, continuous hemofiltration has become the standard of care around the world for acute renal failure associated with multiple organ failure in the intensive care unit. It is now used in a pumped venovenous mode to permit full nutrition and protein support with standard nutritional formulations. Our patient TE was in renal failure secondary to his septic shock and was managed with continuous hemofiltration with the hemofilter simply attached to the ECLS machine.
Nutrition in critical illness
One of the basic problems with supporting critical care patients on life support is how to provide adequate nutrition. The use of mechanical devices to substitute for the gut has been standard practice in our intensive care units and our surgical practices for many years, providing protein and caloric sources through a catheter with direct access into the right atrium of the heart. Francis Moore, my chief of surgery, defined the basic science of body composition, fluid compartments and nutrition in the 1950s and 1960s. In his classic book Metabolic Care of the Surgical Patient, he made the medical community familiar with balance diagrams and the metabolic response to injury. In that book he postulated that if it were simply possible to give enough protein and carbohydrate feeding, it might be possible to reverse the negative caloric and nitrogen balance in critically ill patients, but he did not know how to do this. At the University of Pennsylvania, residents Stan Dudrick and Doug Wilmore, along with their colleagues and chief Jonathan Rhodes, solved the problem by showing that infusion of very hypertonic glucose directly into rapidly flowing blood did not lead to thrombosis and in fact could produce complete and total nutrition via a parenteral route. Other surgeons, including Arnold Coran, demonstrated that lipids as well as carbohydrates could be used as the energy source.
In the intensive care unit we studied the effect of parenteral nutrition (intravenous feeding) on outcome in critically ill patients with multiple organ failure. We found that multiple organ failure patients in positive caloric balance during their ICU stay had a 90 percent survival, and patients who had a 10,000 calorie deficit had a 20 percent survival. Although this finding is not surprising today, at the time it was valuable to see data documenting the outcome of feeding and nutrition in the ICU. To evaluate the importance of the timing of feeding critically ill patients, we compared patients who were in positive caloric balance on day seven in the ICU compared to patients who were in negative balance on day seven. (To be in positive balance by day seven requires beginning parenteral or enteral feeding by day two of critical illness.) The results were that the patients who were fed early had a better survival rate than those patients who were fed later in their course.
Today, standard practice is full nutritional support for patients with critical illness and multiple organ failure beginning very early in their course in the ICU. Like all temporary organ substitutes, intravenous feeding (the artificial gut) can be lifesaving but is not as good as the real gut. We give as much of the feedings as possible into the stomach or intestine (enteral nutrition). TE was begun on enteral and parenteral feedings on his second ICU day.
Artificial liver
Until 2000, when severe liver failure ensued we had no way of managing those patients short of emergency transplantation. Now we are beginning to investigate mechanical substitution for the liver and reticuloendothelial system. The liver and RES detoxify the blood, removing a variety of metabolically produced products that are toxic to the brain and other organs. The liver also provides the synthesis for new proteins and gluconeogenesis (synthesis of glucose). There are four approaches to mechanical support of the failing liver; the system invented by two nephrologists from Germany, Jan Stange and Stefan Mitzner has the most promise. Stange and Mitzner discovered a way to dialyze off molecules that are bound to protein and not removable by conventional hemodialysis or hemofiltration. They did this by using an albumin-coated hemofilter and dialyzing against a high concentration albumin bath, then "cleaning" the albumin in the bath with a series of sorbent columns. In one study using this device, severe hepatic encephalopathy (the effects of liver failure on the brain) was treated with this device, resulting in rapid and significant improvement in both encephalopathy and liver function. There are more data on more than 500 patients using this device under a variety of conditions of acute liver failure. Our most recent patient was on device for three weeks awaiting liver transplantation, which was subsequently successful.
From laboratory to practice
As in any other combined laboratory and clinical research endeavor, the spin-off from our research has been much more exciting and interesting than the original problem. We have learned a lot about the pathophysiology of critical illness, bioengineering, basic science, the technology of extracorporeal circulation, mechanical ventilation and lung healing. One example of this spin-off is the most difficult step in translating basic research to clinical practice: methods to study life-support devices in prospective randomized trials in which the endpoint is death and the control group is conventional treatment.
This complex problem was addressed at a NIH workshop in 1992 using neonatal ECLS as the example. The research on neonatal ECLS was cited a model of how laboratory to clinical transition should be conducted. Of course, the reason we do all this is not simply to do research and not simply to bring new techniques to the intensive care unit, but to apply this technology to the care of our very sickest patients. Our first neonatal ECLS patient is now 27 and has two children of her own. Bridging basic science to clinical practice was certainly worth it in her case.
Back to our patient TE. He was maintained on extracorporeal circulation for 7 days and on continuous hemofiltration then dialysis for 20 days. He was discharged from the ICU and eventually recovered enough to return to the IU cheerleading squad. In the photo, he is shown carrying the IU flag onto the field at the University of Michigan stadium, where these two teams played in the fall of 2000. Of course, we are very glad that the application of all this basic science research to the development of artificial organs in the ICU had such an outstanding result for TE. However, despite TE's best efforts, Michigan won the football game.
Relevant link
The University of Michigan ECMO Home Page(www.med.umich.edu/ecmo)